Chapter 6 : Water Quality
- 6.1 Introduction
- 6.2 Temperature
- 6.3 Dissolved Oxygen
- 6.4 Carbon Dioxide
- 6.5 Phosphorus and Nitrogen
- 6.6 Sediment Contaminants
- 6.7 Metals
- 6.8 Organic Contaminants
- 6.9 Longitudinal Gradients
- 6.10 Depth Gradients
- 6.11 Water-Quality Management
6.1 Introduction
Flowing water stored in a reservoir undergoes various physical and chemical transformations that can change the quality of fish habitat within the reservoir and the river downstream. The extent of the transformations is related to the water retention time in the reservoir, which is controlled by the reservoir’s storage capacity in relation to size of the watershed and extent of precipitation. Water in small and shallow navigation reservoirs in a large river generally undergo little or no transformation because of short retention time; conversely, water stored for many months or even years behind a deep storage reservoir in a minor tributary may undergo major transformations that can affect most life in the reservoir and in the river below the dam.
Water-quality degradation can vary widely in reservoirs. Dissolved gases and temperature often receive the most attention. Dissolved oxygen is necessary to sustain aquatic life, and temperature regulates biotic growth rates. Both temperature and dissolved gases control other physical characteristics of the water as well as chemical reactions and define the biotic, and more specifically the fish, assemblage that develops in the reservoir. Nutrient enrichment principally with phosphorus and nitrogen promotes excessive primary production, which can deplete oxygen (section 4). Contaminants including organic chemicals and trace metals are of concern because they accumulate within sediment and move through food chains and food webs (Erickson et al. 2008; Stahl et al. 2009). Total dissolved solids concentrations may be of interest for water supply and other uses. Turbidity is also a key water-quality characteristic because its effects on light transmission and water clarity define habitat characteristics (section 5). A major aspect of turbidity is total suspended solids, which are also a major transport and deposit mechanism for nutrients and contaminants in reservoirs (section 4). Water pH regulates aquatic chemistry, which can affect water use and habitat. The reservoir hypolimnion is often low in dissolved oxygen and can accumulate dissolved phosphorus, iron, manganese, and sulfide, which can produce water-quality problems within the reservoir and downstream if hypolimnetic water is upwelled or discharged. Upwelled phosphorus (internal loading) can cause summer-time algal blooms. Iron and manganese affect water color and can produce water treatment problems when water is withdrawn for municipal uses. Sulfide causes odor problems when it escapes during reaeration.
Water quality in reservoirs often can be controlled by depth-selective withdrawals. However, in many cases discharges create water-quality problems downstream in the tailwater (Miranda and Krogman 2014). Water-quality management alternatives include management techniques that can be implemented in the watershed before potential pollutants reach the reservoir (sections 2 and 8) and management techniques that may be applied within the reservoir. Other in-lake management practices include phosphorus inactivation and sediment oxidation, biomanipulation, hypolimnetic aeration, artificial circulation, and sediment removal and are discussed below.
6.2 Temperature
A reservoir’s annual temperature regime is perhaps the most important water-quality attribute, capable of influencing various other water-quality characteristics. Thus, knowledge of the temperature regime is key to water-quality management. Vertical thermal variation in the reservoir produces density stratification (Elçi 2008). In the less dense epilimnion, temperature decreases slowly with depth as this upper layer is usually influenced by the depth to which light penetrates. The metalimnion separates the epilimnion from the more dense deepwater hypolimnion. In the metalimnion there is a thermocline where temperature changes rapidly with depth. Below the metalimnion, in the hypolimnion, temperatures again change slowly with depth.
The physical properties of water contribute to this temperature-induced density stratification (Wetzel 2001; Elçi 2008). Because water warmer than 39°F (4°C) is less dense, the warmer waters usually exist near the surface. But because water colder than 39°F is also less dense, some temperate reservoirs have colder waters at the surface mostly during the winter. Under both of these conditions, a reservoir may be thermally stratified. During the fall, the reservoir cools at the surface and experiences cooler inflows. At the surface the cooling produces shallow instability, and mixing can occur at progressively greater depths. This process continues through early winter. When the reservoir finally achieves, thorough cooling and mixing, a uniform temperature surface to bottom, the reservoir is said to be isothermal. This condition usually occurs in winter and continues until spring. These reservoirs experience one season of mixing each year (i.e., monomictic reservoir).
Besides water temperature, water density is also determined by dissolved substances in the water (Wetzel 2001). Increased dissolved solids increase water density. In reservoirs, dissolved solids are influenced by natural watershed characteristics and by anthropogenic activities in the watershed. In some reservoirs the density of deep waters is high enough that complete mixing does not occur (i.e., meromictic reservoir). Meromictic reservoirs are often deep (Wetzel 2001).
Many northern reservoirs stratify during winter (Rahman 1978). Their surface temperatures will be at or near freezing, and often the reservoir may be covered with ice, with slightly warmer and denser water toward the bottom. Because these reservoirs mix in the fall until they experience winter stratification and then mix again in the spring thaw until they restratify in summer, they have two seasons of mixing each year (i.e., dimictic reservoir). This condition also may occur occasionally during winter in shallow, isolated embayments of reservoirs in more southern temperate latitudes.
However, some reservoirs may experience short-term thermal stratification followed by frequent mixing (polymictic), or not stratify at all. Shallow depths, short retention times, and extensive wave-induced mixing may prevent stratification. If no stratification occurs, then the reservoir may consist exclusively of an epilimnion with possibly a weak thermal gradient, and the reservoir consists primarily of warm-water conditions.
6.3 Dissolved Oxygen
Next to temperature, dissolved oxygen is another key indicator of reservoir water quality. The concentration of dissolved oxygen in the reservoir affects the capacity of inorganic substances to reduce other substances as well as the distribution of aerobic and anaerobic organisms. The dissolved oxygen demand may be classified into sediment oxygen demand and water column demand (Cross and Summerfelt 1987).
Sediment oxygen demand is the rate of oxygen consumption by bacteria and other organisms that metabolize organic matter in the sediment. Sediment oxygen demand is usually greatest in the uplake region of the reservoir, or in the entrance to embayments where most sediments are deposited and water is warm and shallow (section 3). Organic matter in sediment in these upper regions is high, and hypolimnetic dissolved oxygen can be depleted, particularly given that the volume of water is typically small because the uplake regions of a reservoir are often shallow (USACE 1987c). Nevertheless, these upper regions do not always stratify because their shallow depth may allow mixing by wave action and sometimes by limited flows.
Water column oxygen demand is the rate of oxygen consumption by bacteria and other organisms that metabolize organic matter in the water column above the sediment. Density flows transport oxygen-demanding material into the metalimnion and hypolimnion and entrain reduced chemicals from upstream anoxic areas to augment water column oxygen demand. Organic compounds, including those in dead organisms from the epilimnion, settle more slowly in the metalimnion and hypolimnion because of increased water density caused by lower temperatures. Because this organic matter can remain in the metalimnion and hypolimnion for a long time, decomposition occurs over a long period, exerting long-term oxygen demands (USACE 1987c).
A threshold concentration of 4–5 ppm often is used to set dissolved oxygen water-quality standards. Hypoxia results when dissolved oxygen concentrations fall to less than 2 ppm, which is generally accepted as the minimum level required to support most animal life. When dissolved oxygen levels become severely depressed or anoxic (near 0 ppm), anaerobic conditions occur. Although anaerobic conditions typically occur in near-bottom waters, it can extend upward through the entirety of the water column. Hypoxia has been shown to be an endocrine disrupter in fish, which impairs fish reproduction (Wu et al. 2003).
6.4 Carbon Dioxide
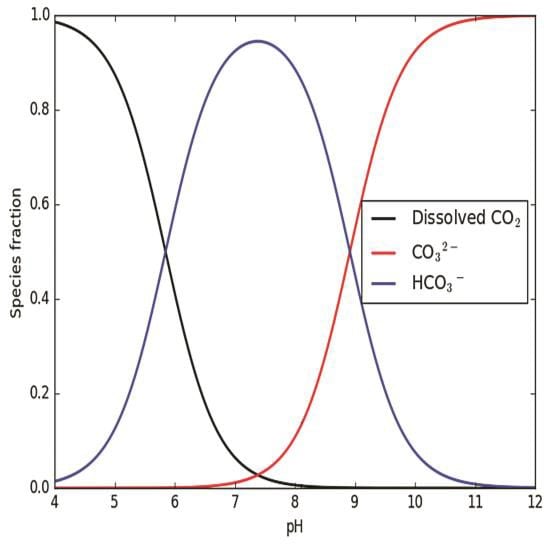
Inorganic carbon derived from carbon dioxide is used by plants to produce organic matter. Inorganic carbon also can control the pH and buffering capacity of aquatic systems. Inorganic carbon occurs in equilibrium in three forms (Figure 6.1): carbon dioxide, bicarbonate ions, and carbonate ions (USACE 1987c). When plants use inorganic carbon to create organic compounds through photosynthesis, the pH goes up, and the concentrations of carbon forms shift from carbon dioxide to bicarbonate ions and to carbonate ions (Wetzel 2001). The scope of this pH rise and shift in the forms of carbon indexes the buffering capacity of the water. A system with low buffering capacity (i.e., with low alkalinity) is likely to have larger fluctuations in pH and shift quicker from carbon dioxide to bicarbonate ions and carbonate ions than systems with higher alkalinity (Wetzel 2001).
6.5 Phosphorus and Nitrogen
Phosphorus is needed by plants and animals to build enzymes and to store energy in organic compounds, whereas nitrogen is needed to build protein. As a gas, nitrogen is important to water quality mostly when there is too much of it (i.e., super- saturated). Supersaturation can cause injury or death of aquatic organisms, including fish. This is not a common problem in most reservoirs, but supersaturation can harm fish below some hydroelectric facilities (Weitkamp and Katz 1980). Phosphorus and nitrogen are typically the key nutrients controlling primary production and are considered in detail in section 4.
6.6 Sediment Contaminants
Sediment plays a significant role in shaping the water quality of reservoirs. Benthic habitats are an environmental sink for many contaminants, as many pollutants sink through the water column to bond with sediment particles (Horowitz 1985; Mulligan et al. 2001). Because sediment is also an important biological habitat, uptake of toxicants into the food web is influenced by toxicant concentrations in sediment. Pollutants that settle out into sediment exist in an equilibrium state with the water above, but this equilibrium may be altered by natural and anthropogenic environmental disturbances (Theofanis et al. 2001; Jaglal 2009).
In polluted waters, pollutants mainly are found adsorbed by particles and bound to organic sediment (Chapman 1992; Baldwin et al. 2002). Changes in environmental conditions alter the various phases of pollutants found on particulates, sometimes causing the pollutants to be released into solution. Various forms of organic matter can be degraded under oxidizing conditions, releasing bound pollutants (Chapman 1992). Particulate pollutants may also become soluble within the acidic digestive tracts of detritivores such as gizzard shad, releasing these substances within the animal and making bioaccumulation through predation possible (Eagles-Smith et al. 2008).
As a result of changing environmental conditions, including dissolved oxygen and temperature, there is an internal recycling of pollutants in the sediment and hypolimnion (Baldwin et al. 2002). The processes are complex and poorly understood. The greatest amount of information is available for mercury and phosphorus. The transfer of mercury from sediment is mediated by bacteria that convert sediment-bound mercury to soluble mono-methylmercury or to volatile di-methylmercury, depending upon the pH (Erickson et al. 2008). The recycling of sediment-bound phosphorus (i.e., internal phosphorus loading) is particularly important because it may increase the rate of eutrophication within a reservoir. Many environmental and physical processes are involved in the release of phosphorus. A common process is the release of phosphorus bound to iron oxide under reducing conditions found in sediment. When bottom waters are anoxic, interstitial phosphate diffuses to the overlying water, increasing the rate of eutrophication.
6.7 Metals
Become a Contributing Sponsor
Become a part of projects that need your support.